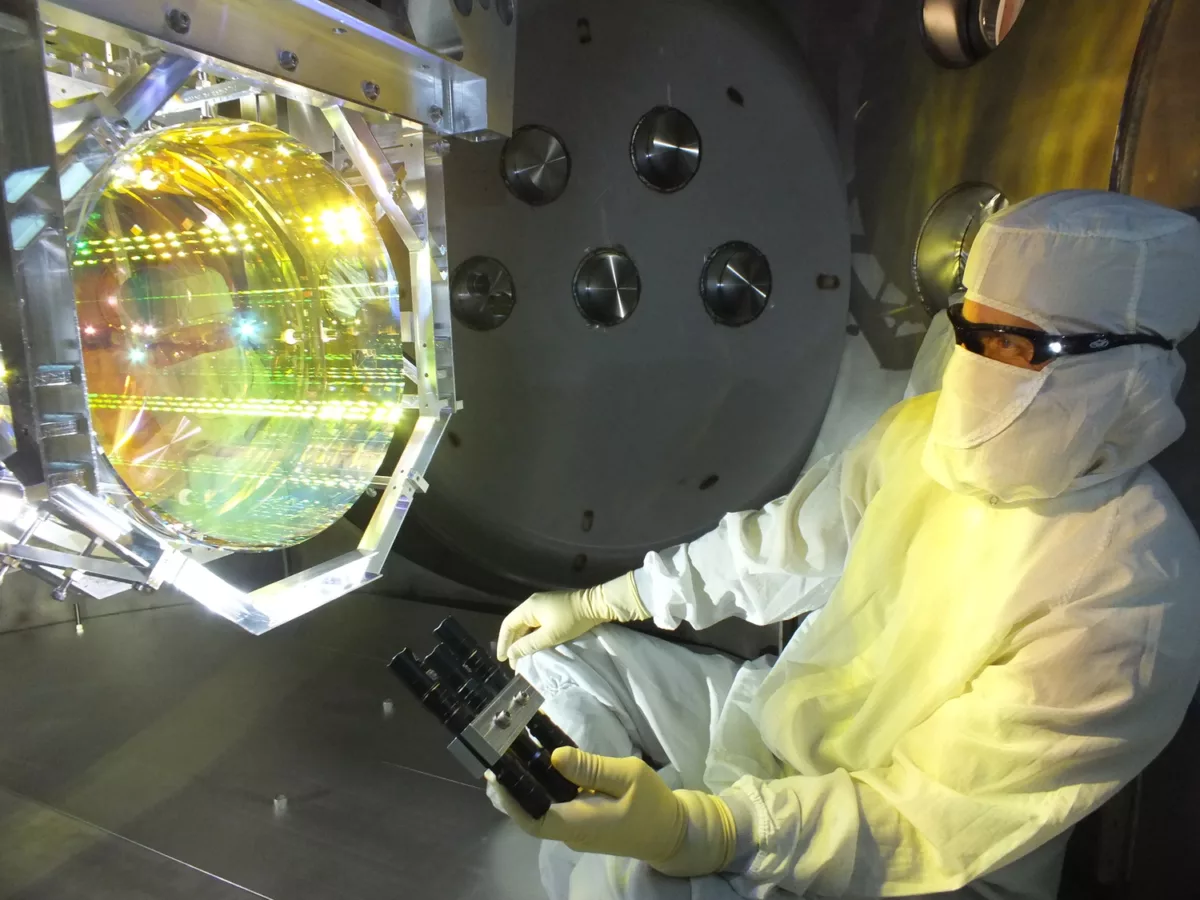
Seismic and geodetic instrumentation are often used to scope out the inner workings of the Earth—things like earthquakes, rock properties, and planetary composition. But these techniques also support and enable other fields of science, including fundamental physics and astronomy. For example, they help researchers filter out seismic noise that interfere with sensitive experiments, such as those detecting neutrinos or gravitational waves, ultimately improving the accuracy of their findings in complex, high-precision studies.
The tip of the iceberg
In Antarctica, scientists use nearly 1 cubic kilometer of the ice sheet to study one of the universe’s most elusive particles—neutrinos.
Neutrinos are cosmic particles originating from processes in the interior of stars like our own Sun, during the violent explosions of stellar death, and even from black holes. These particles are nearly massless, allowing them to travel imperceptibly through almost anything at close to the speed of light. Since neutrinos are so elusive, scientists must concoct clever experiments to study them.
The astrophysical observatory at the South Pole, called the IceCube Laboratory, sits close to the Amundsen-Scott South Pole Research Station and aims to detect passing neutrinos using the ice sheet itself. With plentiful ice and minimal disruption from seismic waves, scientists constructed a large detector to catch a glimpse of these ephemeral neutrinos.
When a neutrino hurls through the ice, it produces secondary particles with an electrical charge that give off light. This light is called Cherenkov radiation—a phenomenon in which light emitted from the charged particle moves faster through the ice than it otherwise would normally. Cherenkov radiation is the smoking gun of a neutrino interaction, and is what IceCube probes the ice for with nearly 600 detectors in an array of 86 boreholes.
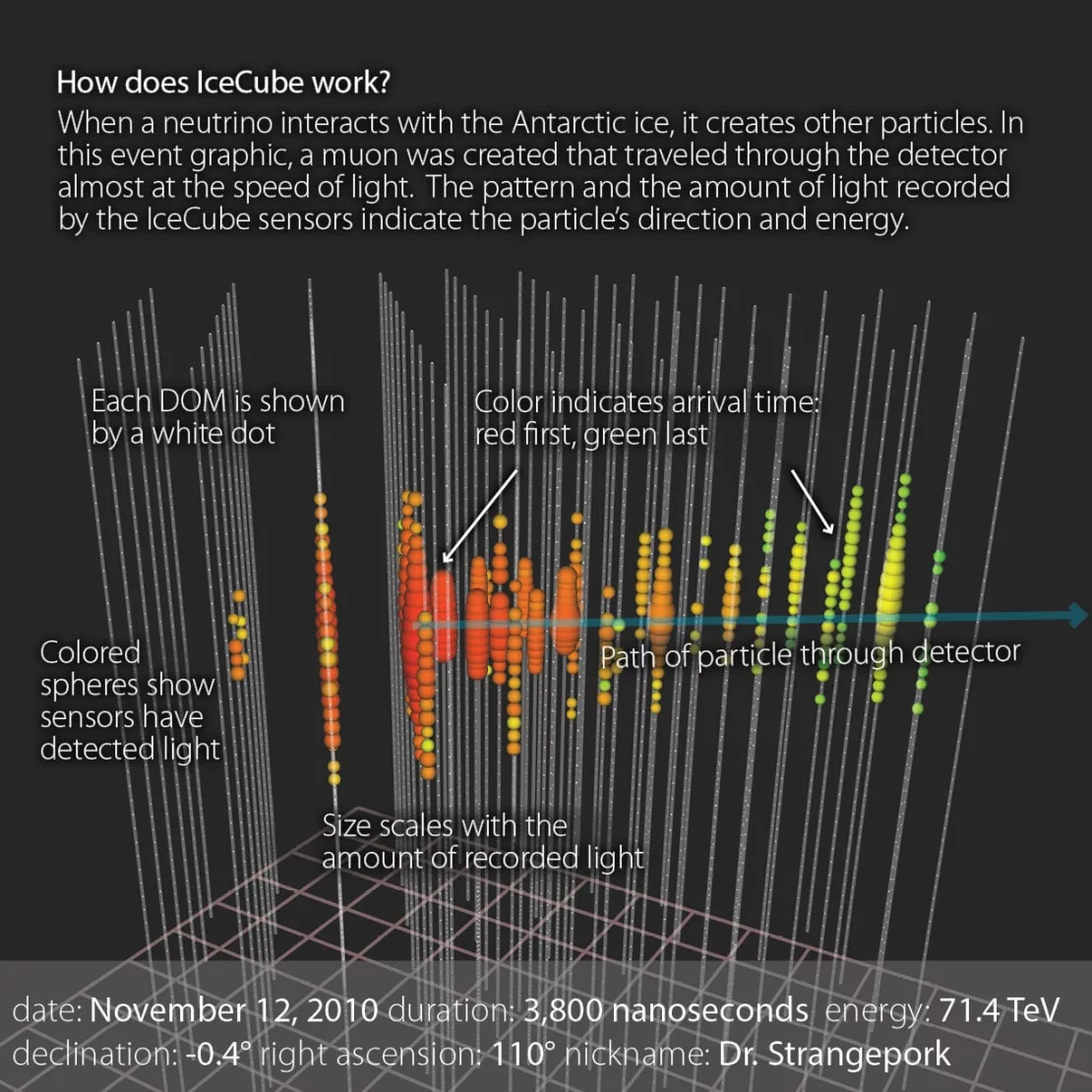
To make sure that the Antarctic ice remains a stable test bed for neutrino science and isn’t deforming while IceCube tries to make measurements, tiltmeters( a type of geodetic instrument that measures, well, tilting) were installed into the ice alongside the array. GPS instruments were additionally included to provide accurate timing of for IceCube’s measurements of neutrino bombardments. With so many similarities to geophysical monitoring stations, it is no surprise that IceCube has the potential to work alongside the nearby Quiet South Pole Antarctica (QSPA) station in the Global Seismographic Network. Researchers hope to install a broadband seismometer within a deep IceCube borehole for an exceptionally quiet station. Installation of these instruments alongside IceCube will allow geophysicists to probe Earth’s interior in greater detail while simultaneously studying the universe’s most elusive particle.
Since coming online in 2011, IceCube’s cutting-edge approach has helped identify neutrinos originating from events far beyond our home in the Milky Way Galaxy. Neutrino detections from IceCube complement astrophysical observations of black holes and supernovae from other instruments, offering a crucial line of evidence that enhances understanding of these cosmic phenomena.
A ripple effect
Kilometers below the surface in the Black Hills of South Dakota, the western hemisphere’s largest and deepest gold mine has been converted into an underground laboratory for geology and astrophysics. The Homestake Mine, once a significant producer of gold, is now known as the Sanford Underground Laboratory. Here in the 1960s, one of the first underground neutrino detectors was established to understand the cosmic abundance of neutrinos. The successful discovery of neutrinos produced by nuclear fusion in the Sun led to a flurry of further exploration to understand more about different types of particles in the cosmos.
Now in 2025, further excavation of the Homestake Mine is making way for a new neutrino detection experiment. The Deep Underground Neutrino Experiment, or DUNE, is currently set to complete construction this year. Newer and larger detectors are being installed to detect a stream of neutrinos that will be beamed toward Sanford from a particle accelerator at Fermilab in Batavia, Illinois, some 1,300 kilometers (800 miles) away. Researchers hope to better understand how neutrinos behave by closely following their journey through the Earth.
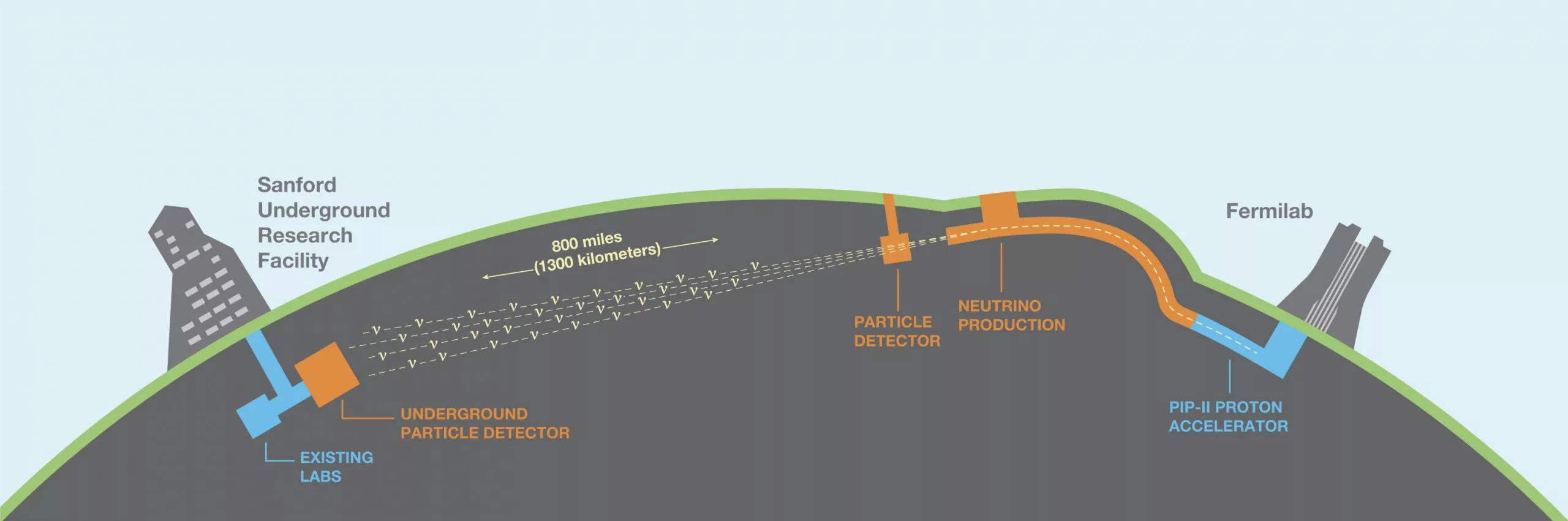
But in order to make this upgrade, researchers had to carefully survey the mine to ensure it was safe to build an entire underground lab. Previous seismic surveying to characterize the locale, the temperature-driven deformation of the rock, and even humidity were carefully accounted for while planning these excavations. Seismic tomography was used to create a 3D visualization of the subsurface prior to construction of the caverns to understand the mine’s rock mechanics. Seismicity is carefully monitored for data correction because the facility sits within the Black Hills, a region that is influenced by active uplift and adjacent tectonic motion, but remains low risk for underground activities.
Einstein was right
Underground physics laboratories provide unique opportunities to test out fundamental theories of the universe, and seismic and geodetic data can inform the best sites. The extensive surveying of the Homestake Mine for experiments like DUNE made the mine an intriguing option for one of the first efforts to detect gravitational waves underground. At Sanford, the Deep Underground Gravity Laboratory or DUGL, initially helped establish a method for detecting gravitational waves from Earth.
Gravitational waves ripple through and distort space-time, originating from astrophysical sources like extremely distant supermassive black holes and neutron stars. When these extremely massive objects orbit one another or merge together, they release gravitational waves which propagate through the universe and eventually reach Earth as nearly imperceptible distortions of spacetime.
Detectors of gravitational waves are like strainmeters for the universe. Similar to strainmeters that are able to detect even the subtlest changes in stress within rocks from Earth movements, gravitational wave detectors can be thought of as much larger and more sensitive strainmeters for the faint stretches and compressions in space-time.
To detect these events, gravitational wave detectors need to span a large area to distinguish the faint signal of gravitational waves passing through Earth from other sources of vibration, like local ambient noise and seismicity. That means the facility isn’t just in one place—one detector is typically located thousands of kilometers away from the next one, creating a type of observatory called an interferometer.
Interferometers are not just used in astrophysics. They are common, large array systems used to detect faint signals of interest. In seismology for example, interferometry can be used to cross-correlate ambient noise at multiple instruments to detect things like changes in groundwater levels, while astronomers can use interferometers as massive telescopes to resolve very distant objects. Gravitational wave detectors function the same way, but are sensitive to a different type of observation.
DUGL worked alongside the Laser Interferometer Gravitational-Wave Observatory, or LIGO. This project is famous for detecting the first gravitational waves emitted by a distant black hole merger. By constructing a detector in the Homestake Mine, researchers wanted to understand if the isolation from the surface, where ambient noise is stronger, would improve the signal-to-noise ratio in detecting gravitational waves. Ultimately, it was found that placing a gravitational wave detector underground did little to combat these other signals, but DUGL did pave the way for more sensitive detectors and has even inspired more efforts such as the Cosmic Explorer—which, currently in the planning stage, is taking advantage of USArray data to help evaluate locations.
The detectors that now make up LIGO are split between the Hanford Nuclear Reservation in Washington State and the Livingston Observatory in Louisiana. The distance between these two sites is just over 3,000 kilometers (1,800 miles), but if one gravitational wave is detected at Hanford, it will be detected only 10 milliseconds later at Livingston. Careful alignment and maintenance of these detectors using GPS instruments ensures that a registered signal at Hanford can be correlated with one in Livingston.
The needle in the haystack
LIGO functions by constantly monitoring seismicity, which allows for seismic waves and signals to be subtracted out of the actual readings that may contain gravitational waves. Because it needs to detect gravity fluctuations on the order of 10-9 meters, LIGO is incredibly sensitive—so sensitive that it’s more than capable of picking up environmental noise like weather, traffic, and earthquakes. To keep these instruments as motionless as possible, LIGO employs a seismic isolation system that functions via active and passive vibration absorption.
Passive isolation is achieved by suspending the detectors within a quadruple-pendulum system, which serves to dampen the external environmental motion. The active vibration isolation system is made up of internal isolation platforms that actually move to counteract the local vibration measured by seismometers, similar to the way noise-cancelling headphones remove the sound around you. This leaves the gravitational wave detector undisturbed enough to detect the extremely subtle signals LIGO is after.
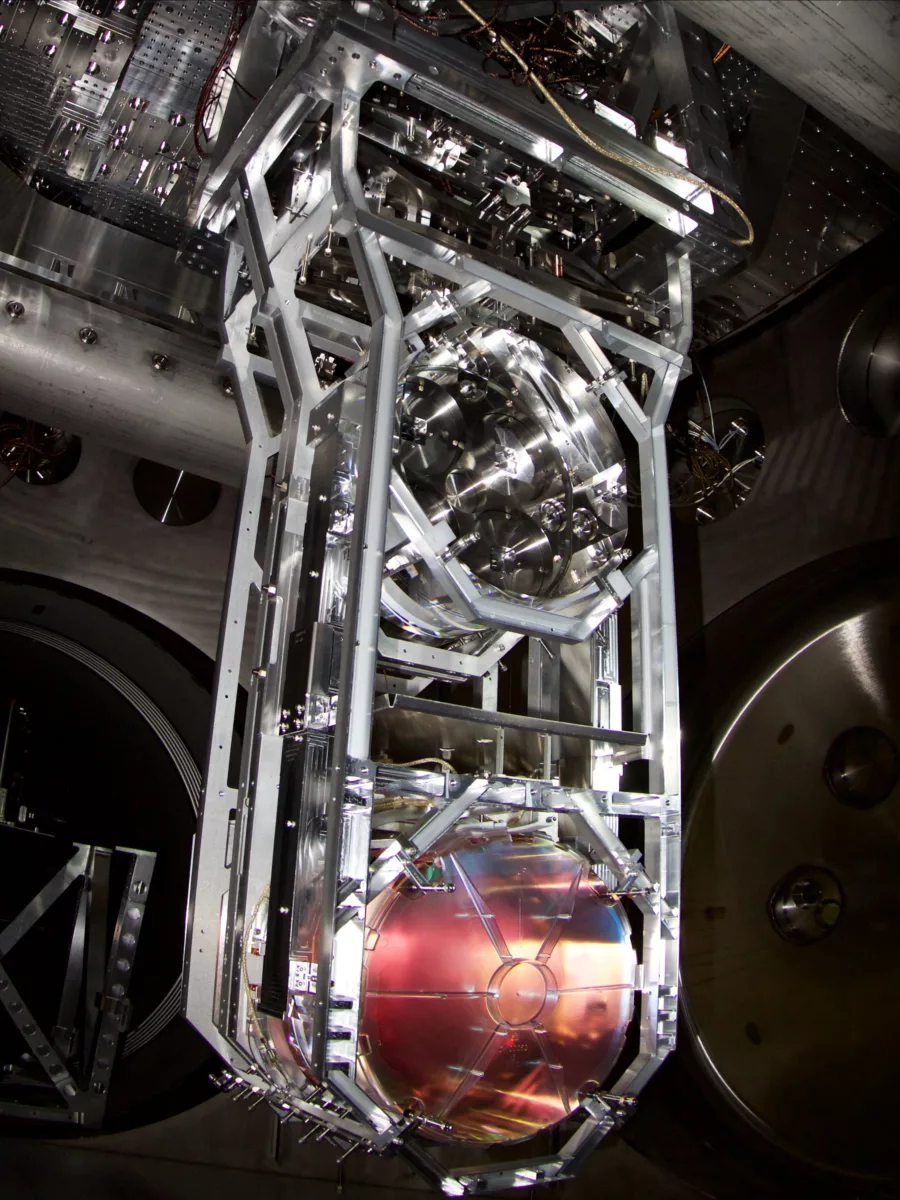
Without the methodologies and instruments pioneered in geophysics, researchers in physics and astronomy would not be able to make key observations to help discover more about the universe. Utilizing the physics of an ice sheet to detect rare cosmic particles, or repurposing the environment of an underground mine to minimize seismic disruption of gravitational wave detection are just a few ways that geophysical techniques are supporting fundamental research. These developments illustrate how cross-disciplinary connections drive progress in scientific discovery, enabling us to uncover insights that were once out of reach.